From Earth is a Nuclear Planet by Mike Conley (Author), Tim Maloney Ph. D. (Author), Stephen A. Boyd Ph. D. (Scientific Editor)
Author: THE KERNEL
Listen to the audiobook here.
Nuclear power’s primary mission is to ensure a reliable and sustainable supply of clean energy for the nation and the world by replacing fossil fuel with the smallest and cleanest possible footprint. A key part of this process is to wean the world off coal by producing energy cheaper and cleaner than coal. Nuclear is already doing this; it just needs to be doing a lot more of it, and in a lot more places. [1]
For nuclear to be a genuine long-term solution, its sustainability model must include the reduction or (much better) the elimination of uranium dirt mining, coupled with the freedom to recycle “spent” fuel rather than sending all of it to long-term storage (see Chapter 18).
The good news is that uranium mining can be significantly reduced, or even eliminated, through any combination of these five technologies:
• Exploit our depleted uranium as fuel for fast-neutron reactors.
• Recycle “spent” nuclear fuel in fast-neutron reactors.
• Breed new fuel from feedstocks of natural uranium and thorium
.• Reprocess used fuel for another round in light-water reactors.
• Extract uranium from seawater to fuel existing and future reactors.
Reprocessing used fuel for a second run in light-water reactors, like France has been doing for the last forty years, is the low-hanging fruit that could cut uranium mining waste nearly in half. After its second run, the twice-used fuel could then be processed and used again in fast-neutron reactors for complete burnup. The alternatives listed above could all be in widespread use by midcentury.
Before we dig any deeper into the subject of energy derived from fuel, we should take a moment to refresh those cherished high school memories of ions and isotopes, and the difference between chemistry and nuclear physics. But this time, let’s do it with lots of pictures and (almost) no math.
First off, you can think of isotopes like cars—identical models of the same sedan, but with different engines. Hydrogen is a simple example: Most hydrogen atoms have nothing but a single proton in the nucleus, and that’s it. When people refer to hydrogen, this is the isotope they’re thinking of: protium, or hydrogen-1. Think of it as the Protium, hydrogen’s base-model ride.
Some hydrogen atoms have a neutron in the nucleus to keep the proton company—that’s the deuterium model. Tritium, or hydrogen-3, is a rare, limited-edition hydrogen isotope sporting twin neutrons under the hood. But so long as it just has one proton, it’s only a hydrogen sedan. (Your neutrons may vary.)
Chemistry is all about the electrons, and does not involve the nucleus of any atom. All chemistry does is rearrange the electrons orbiting the atoms involved in the chemical process. Electrons are negatively charged, and the protons in the nucleus they orbit are positively charged.
An ion is a custom version of an atom’s basic model. The ionization process offers a variety of trim packages that give the atom a greater or lesser number of electrons whizzing around its nucleus in a cloudy blur. Changing the atom’s electron count will give it a formal minus-charge, or a formal plus-charge, depending on whether electrons were gained or lost in the process.
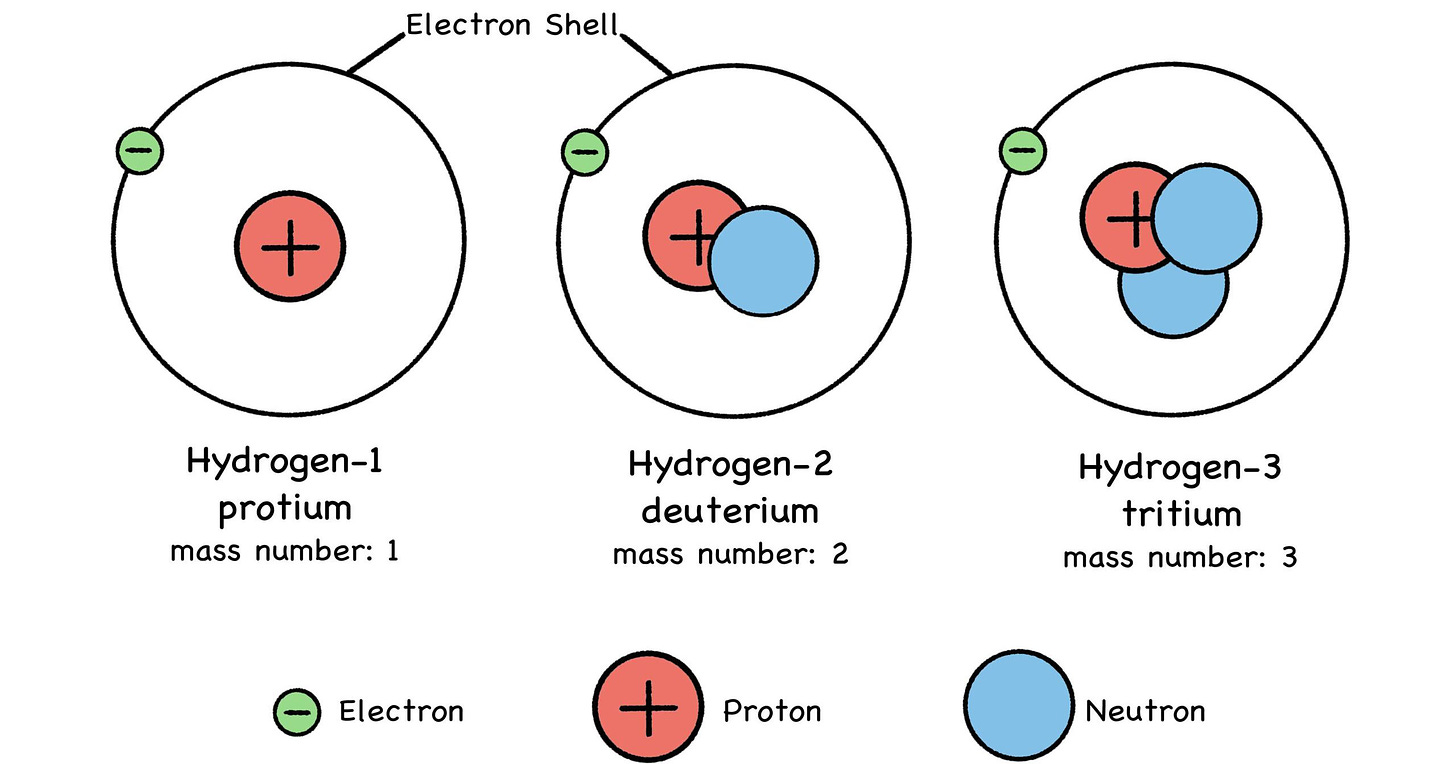
Fig 26: Isotopes of Hydrogen
Hydrogen, for example, typically has one electron orbiting a solitary proton; this protium atom becomes a free-flying proton when its electron is stripped away. That’s how the sun, our first operating fusion reactor, showers us with cosmic rays.
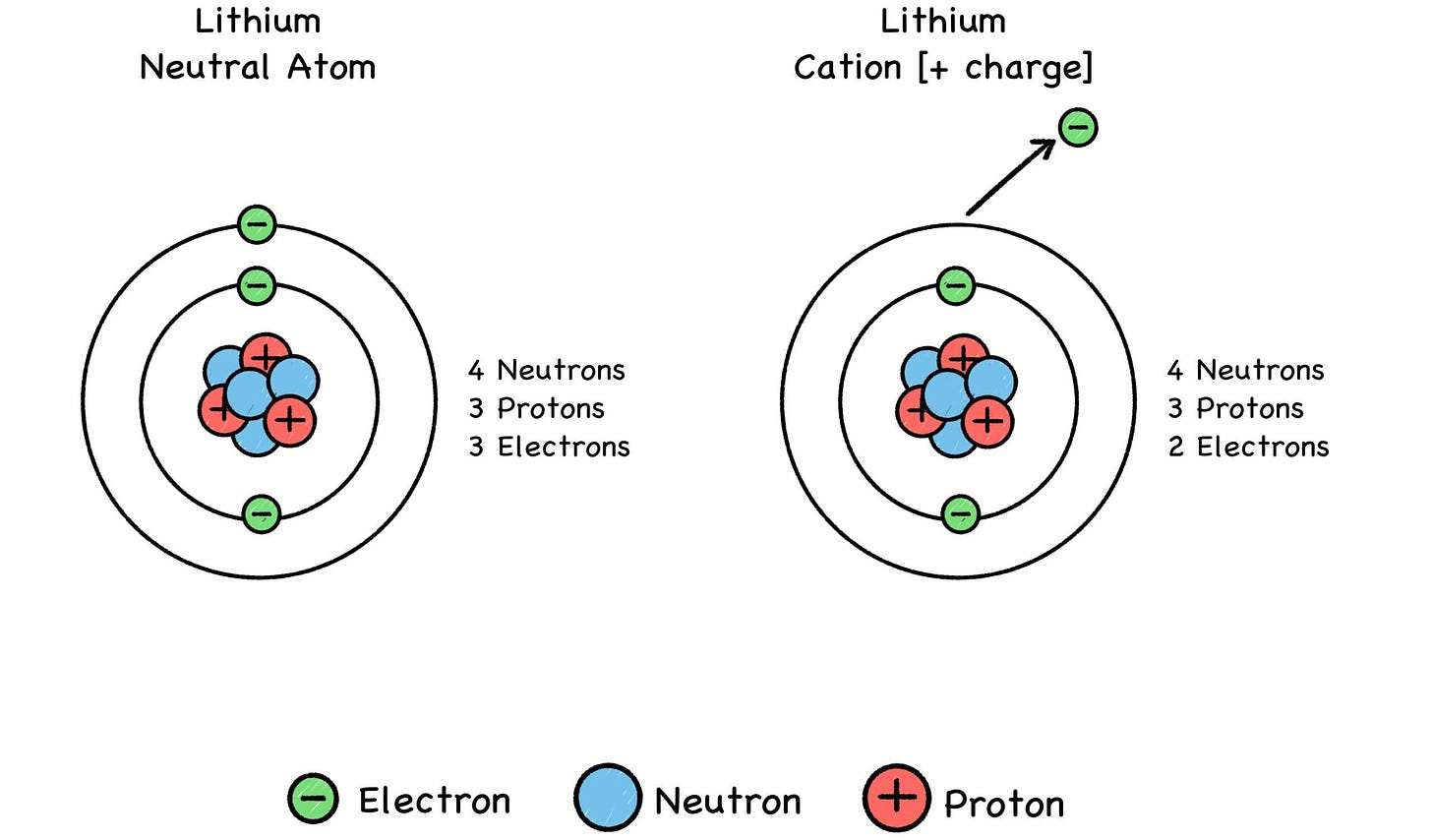
Fig. 27: Positive Ions (“CAT-eye-ons”)
Metals (such as lithium) tend to lose electrons and become cations, and some elements like fluorine tend to gain electrons and become anions. A chemical reaction can alter an atom’s net electric charge by removing or adding electrons, turning the atom into a positive or negative ion of its former self. This can make it attract or repel another atom, depending on the other atom’s charge.
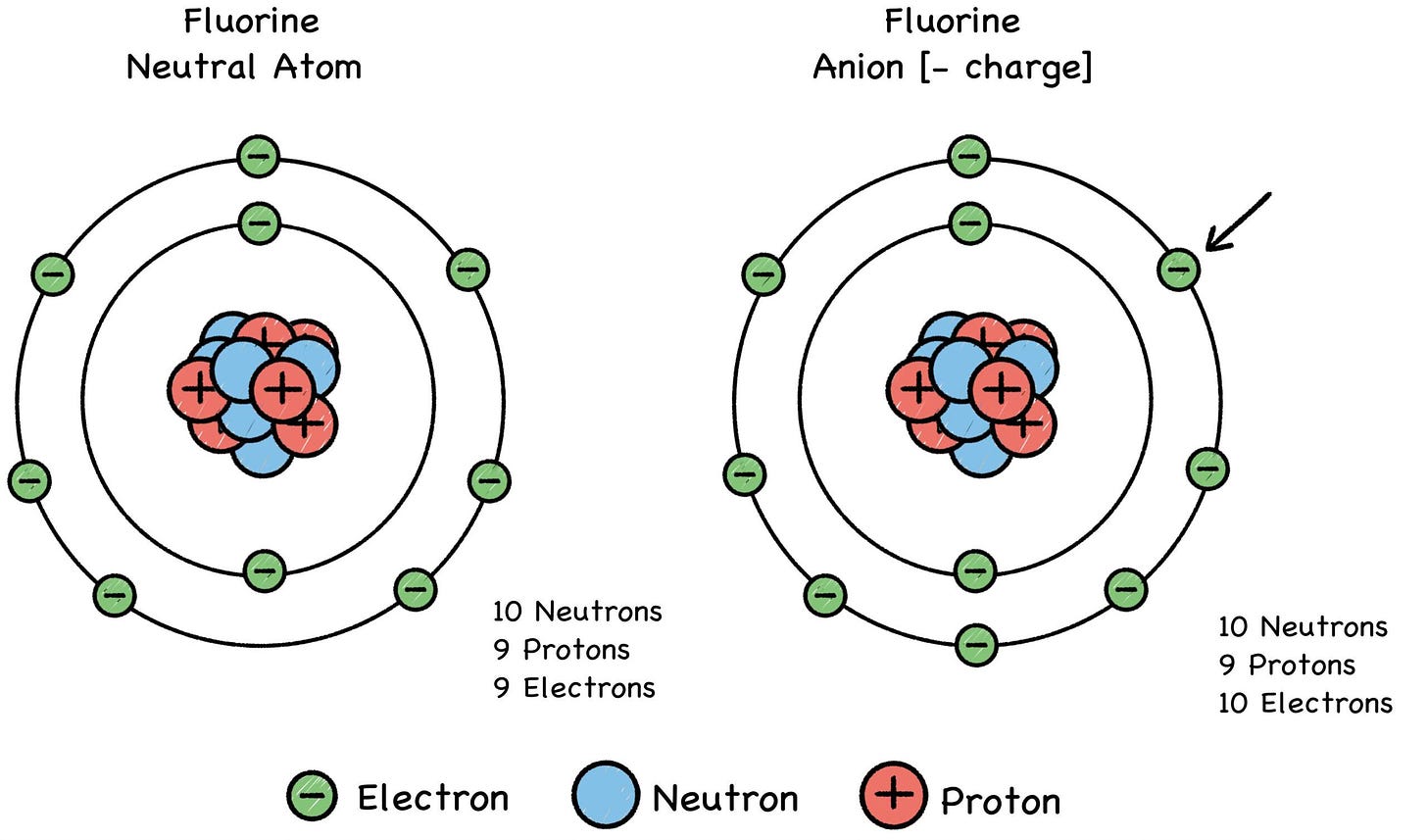
Fig. 28: Negative Ions (“AN-eye-ons”)
Think of ions as two servings of the same ice cream, but for some reason you’re attracted to one and repulsed by the other.
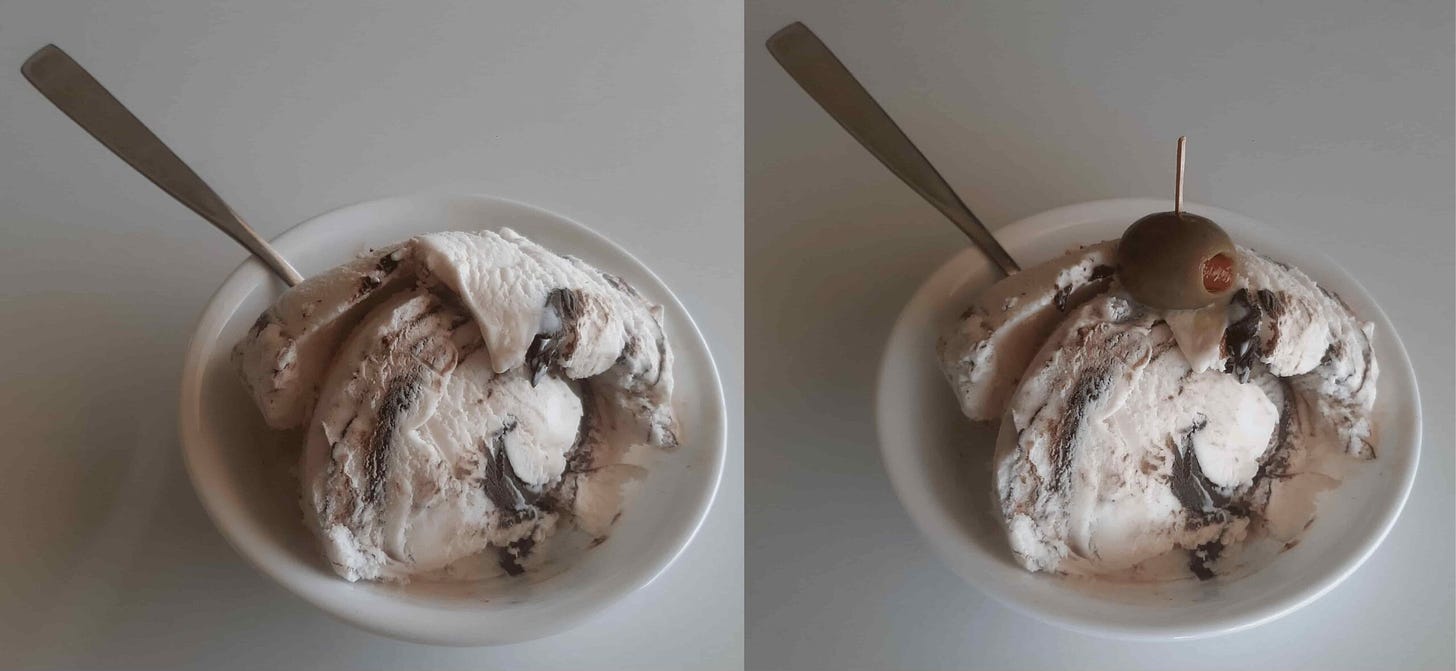
(Pg. 66) Positive and Negative Ions
(We’re sorry you had to see that.)
Chemical energy comes from breaking and forming electron bonds between the atoms involved in a chemical reaction. The process either gives off energy (exothermic), or absorbs energy (endothermic), but either way the field of chemistry is all about the electrons.
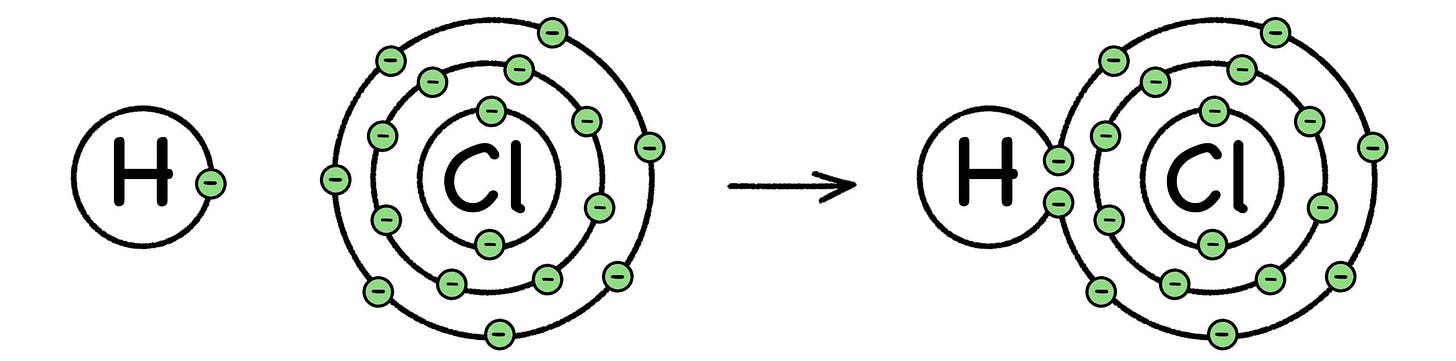
Fig. 29: Formation of Hydrogen Chloride
Nuclear energy, as the name implies, is all about the nucleus, and is typically produced by splitting a uranium or plutonium nucleus with a free-flying neutron zooming along at just the right speed. Because it has no charge, a neutron can zoom undeterred through a thick cloud of electrons and strike a target nucleus.
The neutron could be absorbed, but if it splits the nucleus instead, some of the binding energy that held the nucleus together is suddenly released. Some fast-flying neutrons will zoom away from this high-energy event as two new smaller atoms are formed (sometimes three), with most of the original electrons settling into orbitals around the new atoms. [2]
Zooming neutrons, whether fast, slow, or in between, are the projectiles that crack open the nuclei of heavy atoms—which, conveniently enough, eject one or more zooming neutrons as they split into smaller atoms. And thus we have controlled chain reactions. But not to worry: It is impossible for a reactor to have a runaway chain reaction like a bomb. Fuel enrichment levels are far below the required concentration, at 4%–19% versus 85% or higher for nuclear weapons.
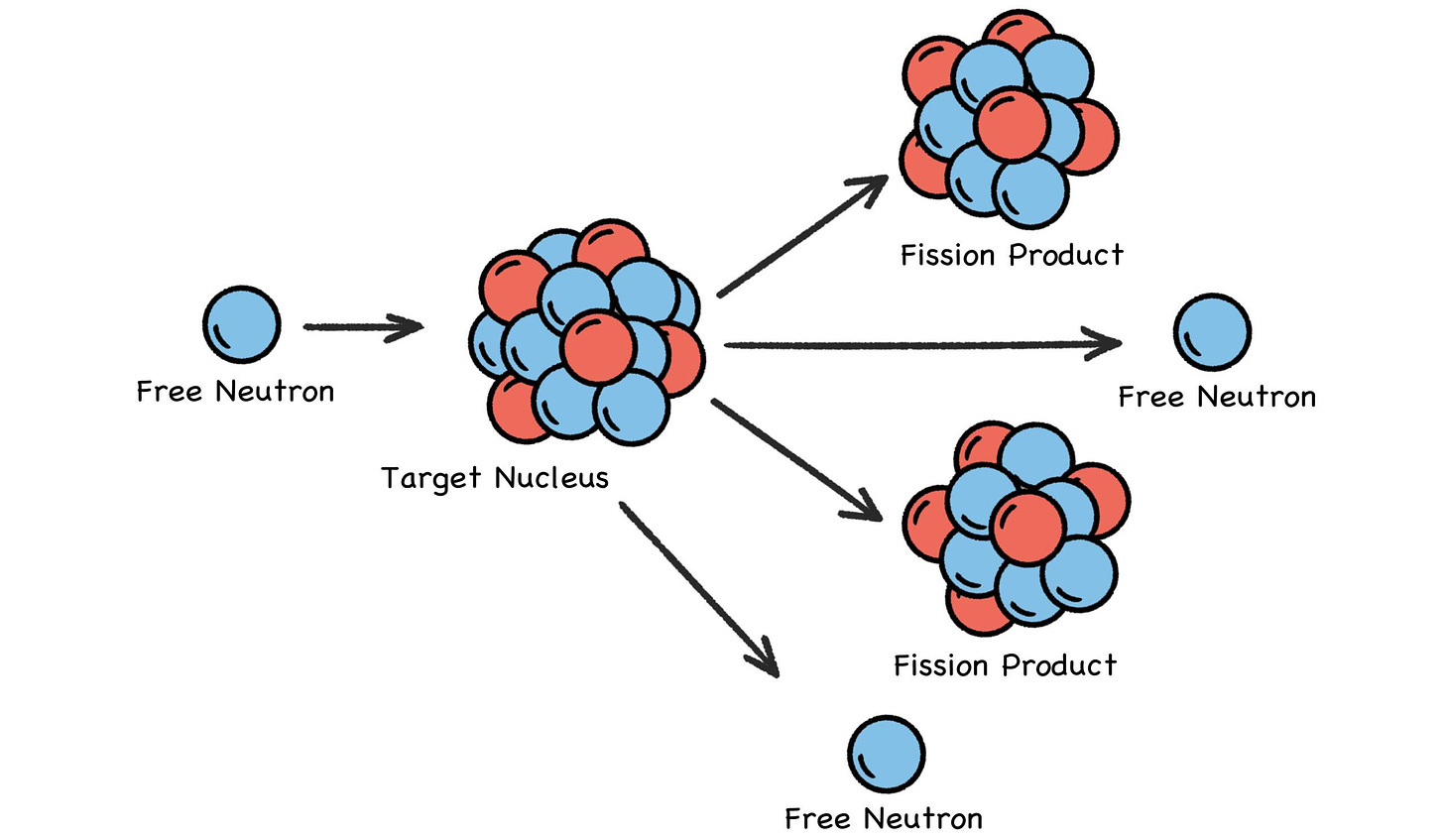
Fig. 30: Nuclear Fission
The energy we get from combustion (wood, coal, diesel, etc.) comes from the sudden rearrangement of electrons when carbon fuel atoms and oxygen atoms react (ignite, combust, explode, etc.). The energy derived from fission comes from splitting some of the largest atoms in the universe. Think of it like this:
The electrons of a large fissionable atom are like a swarm of TIE fighters protecting the Death Star. Chemistry is when the TIE fighters engage in a dogfight with the X-Wing fighters of the Rebel Alliance fleet hovering nearby. Nuclear is when Luke obliterates the Death Star with a proton torpedo down the thermal exhaust vent.
Except nuclear fission uses neutron torpedoes, and the nucleus doesn’t get blown to smithereens. It just breaks into a pair of smaller atoms (sometimes three), and one or two neutrons (sometimes three) go zooming away as well, along with the release of a stupendous amount of energy. But you get the idea.
5.1 MEANWHILE, BACK ON PLANET EARTH
To make fuel pellets for a light-water reactor, uranium is chemically extracted from uranium-bearing ore. The resulting yellowcake powder is purified natural uranium, a metallic powder that’s 99.3% U-238 oxide and 0.7% U-235 oxide.
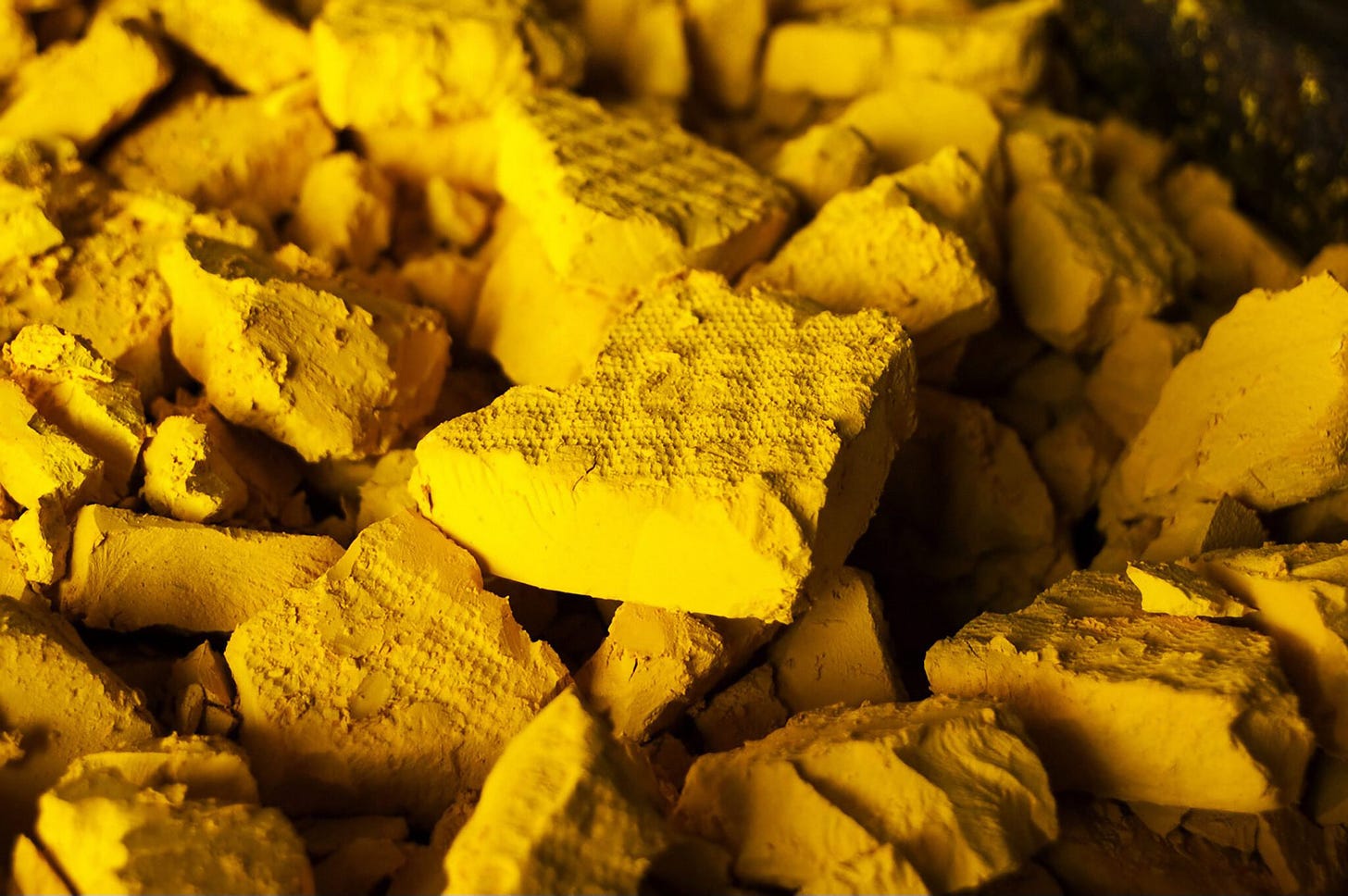
Yellowcake
Credit: US Nuclear Regulatory Commission (CC-BY-SA-2.0)
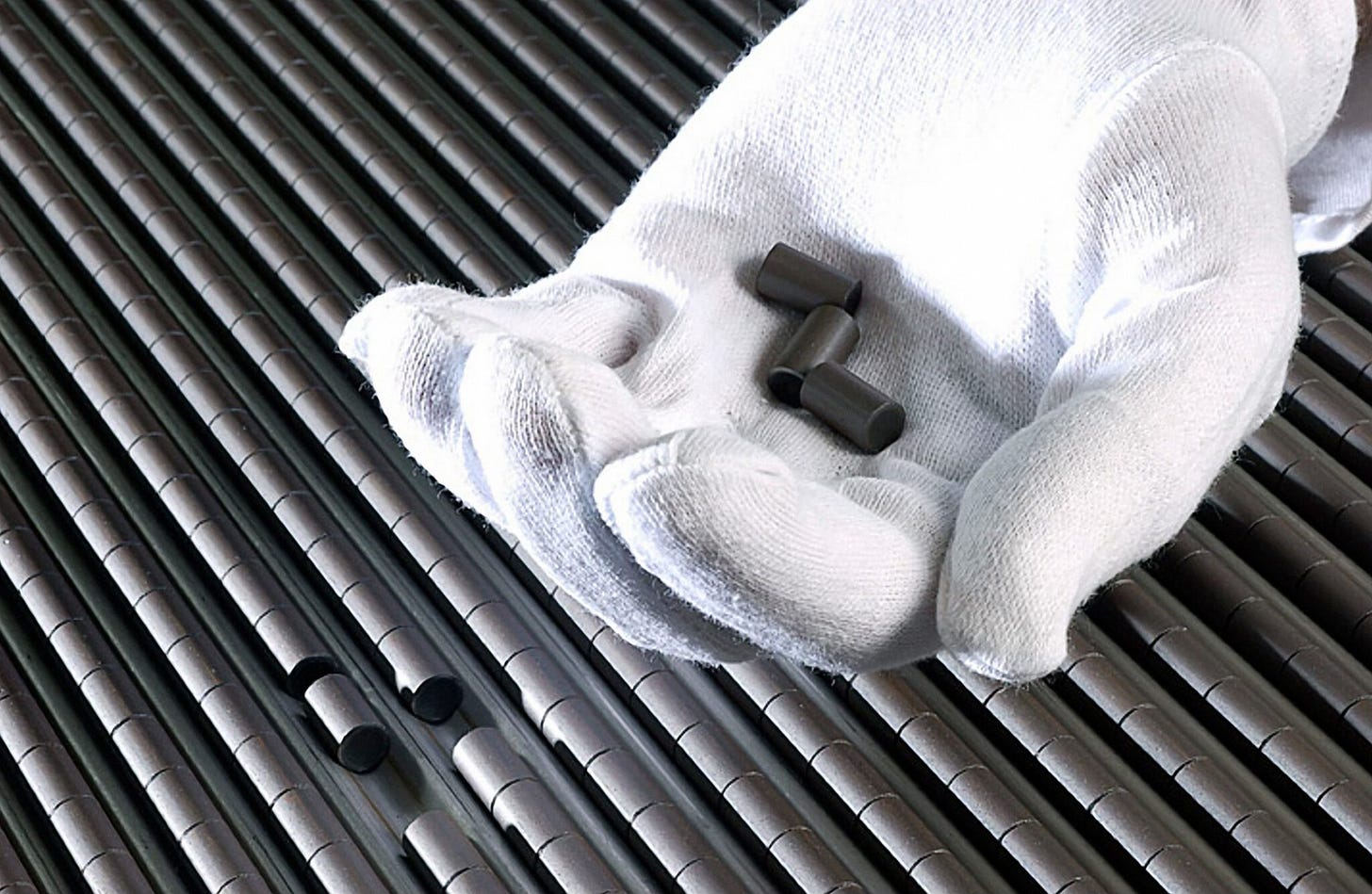
Fuel Pellets
Credit: US Nuclear Regulatory Commission (CA-BY-SA-2.0)
While uranium-238 will not fission, U-235 will, and this is best accomplished with a slow-moving or “moderated” neutron. Any neutron is fast and furious when it zooms away from a fission event, but maintaining its speed (and thus its energy) depends on what it bumps into. Conveniently, water molecules do an excellent job of slowing down zooming neutrons to the proper speed for splitting uranium-235.
The idea of fissioning U-235 in a water-moderated reactor, to make steam to spin a turbine to make electricity, was a logical step in early reactor design. The result was the light-water reactor (LWR), a workhorse technology virtually impervious to climate or weather, and capable of generating on-demand energy for about two years straight before refueling.
Plutonium-239 can form when U-238 absorbs a neutron. This is because while U-238 is not fissile, it is “fertile” and can thus be “bred” into Pu-239, which is an excellent reactor fuel. (A bunch of 20th-century guys came up with these terms.)
Though it rarely happens, a bit of Pu-239 can also be formed when the U-235 in fuel pellets absorbs multiple neutrons instead of fissioning. But however it’s made, the heavier plutonium-239 isotope typically requires a fast (unmoderated) neutron to fission.
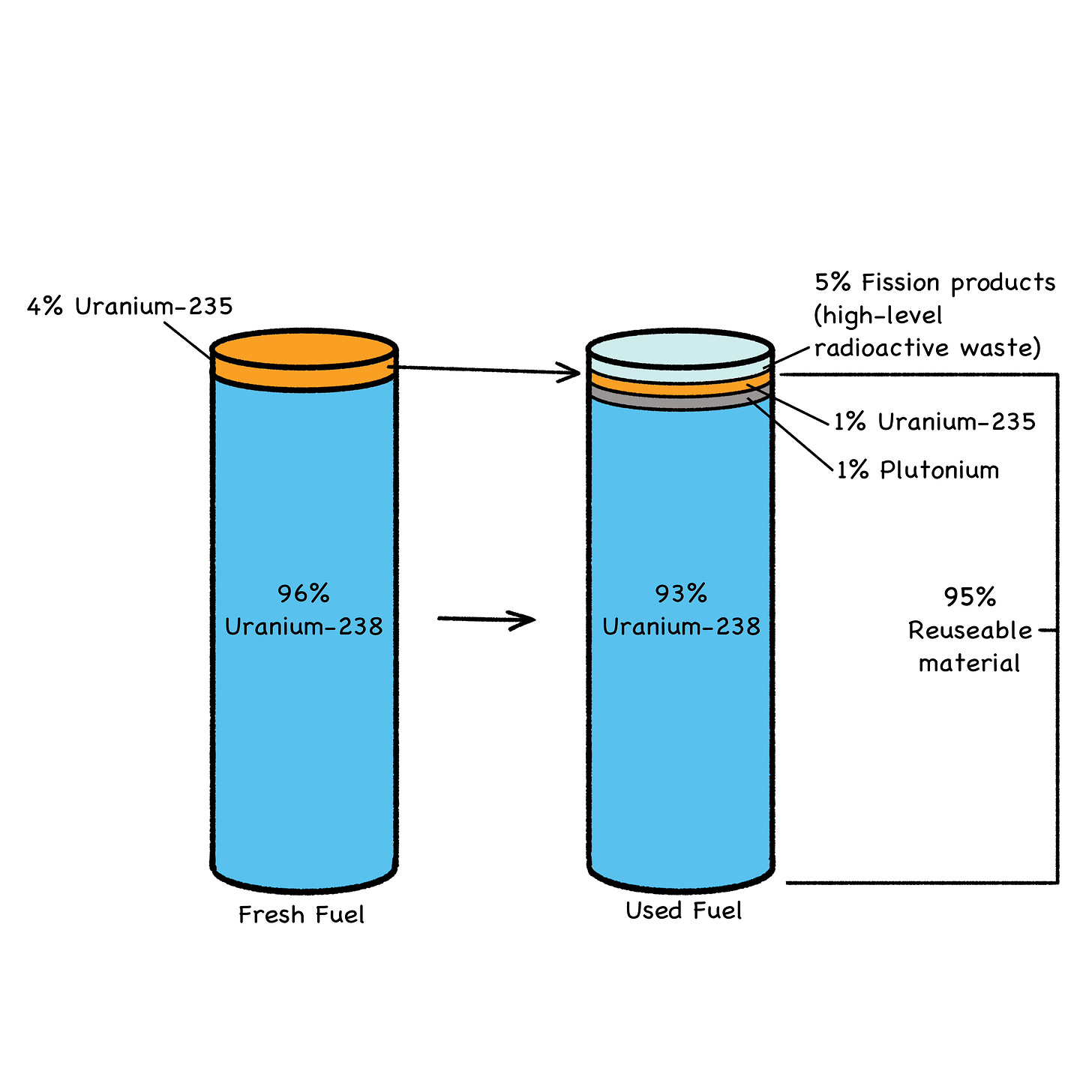
Fig. 31: Used Fuel Makeup
For a number of technical reasons we’ll explore in Power to the Planet, the Pu-239 that forms in a fuel pellet has a 40% chance of fission. But even so, plutonium is such a terrific fuel (it “burns hot”) that more than one-third of the energy produced in a light-water reactor comes from breeding and “burning” plutonium (1950s slang for fissioning nuclear fuel). This is known as the uranium-plutonium fuel cycle. [3]
The remaining 60% of the Pu-239 that forms in a reactor’s fuel pellets will just sit there gobbling up more neutrons like immobilized Pac-Men, turning into larger and larger isotopes: Pu-240, -241, -242, and so on. Higher elements will also be produced, such as ameri- cium-241, the radioactive material used in smoke detectors.
Aside from plutonium-239, however, most self-respecting trans- uranic isotopes wouldn’t even consider fissioning with anything less than a seriously fast neutron. And that’s where complications arise.
5.2 TRANSURANICS HAVE NOTHING TO DO WITH EXPLORING URANUS
Transuranics (TRUs) are the man-made elements that lie beyond (trans-) uranium on the Periodic Table: neptunium, plutonium, americium, curium, berkelium, etc. They’re down in the bottom row, separated from the main table (see Fig. 31 below).
NERD NOTE: The Periodic Table primarily organizes elements by their proton count. All isotopes of uranium, for example, have 92 protons—that’s what makes them uranium atoms. But the Table also organizes elements according to how many electrons occupy their various “orbitals,” arranged as shells within shells like a Russian doll.
Nature has some very clever ways of fitting electrons into these orbitals, but in simple terms, lanthanum and actinium begin their respective rows below the main Table because they are the first elements with f-electron orbitals. Since there can be up to fourteen f-electrons, placing these oddball elements in the Table would make it too unwieldly, so they’re depicted in separate rows below.
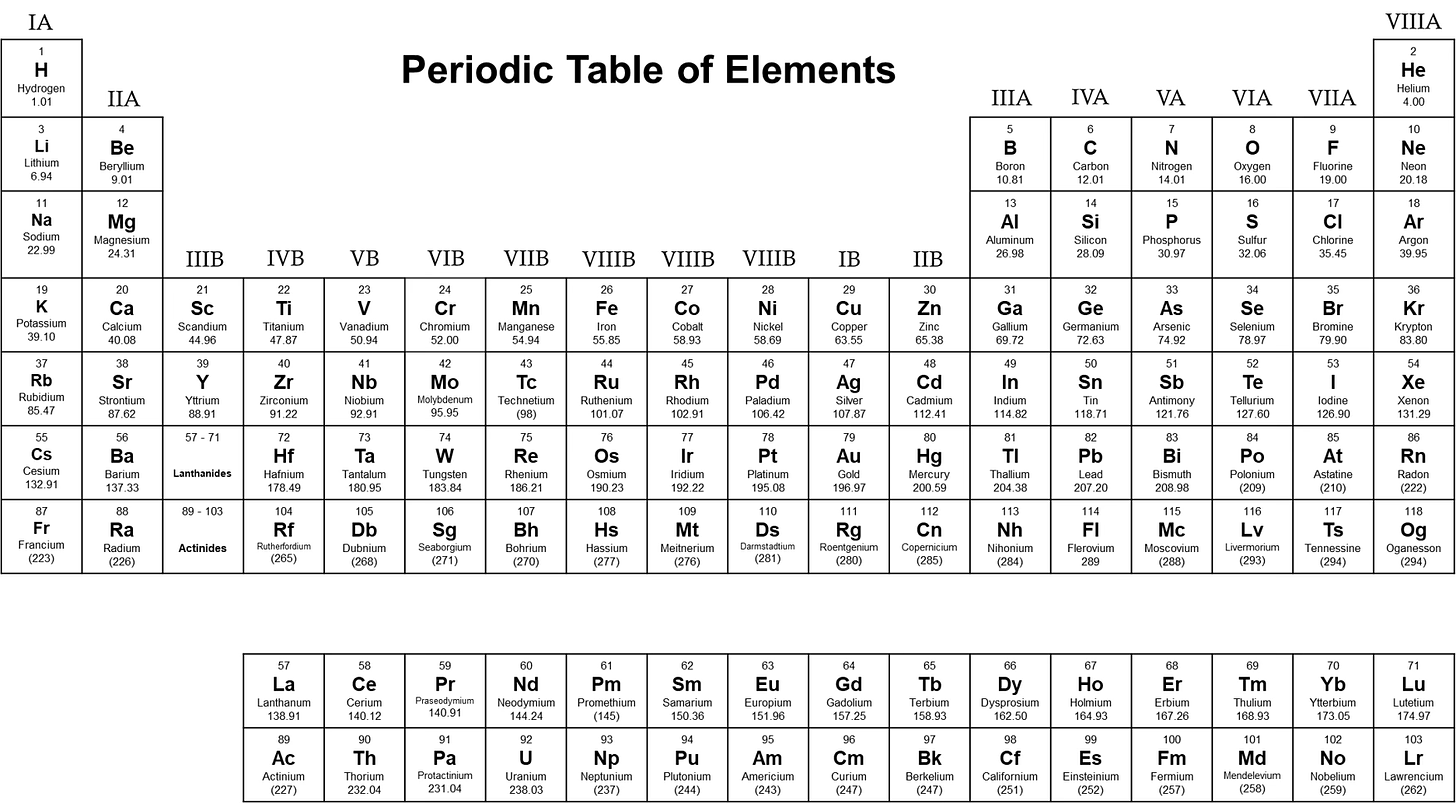
Fig. 32: Periodic Table of Elements
Credit: Emeka Udenze (CC-BY-SA-4.0)
Only a handful of actinide isotopes are long-lived. These trouble- some TRUs come about when the plutonium-239 that forms in reactor fuel absorbs even more neutrons without splitting. It’s these long-lived, man-made isotopes that make used fuel seem like such a vexing problem, even though it’s actually not. For one thing, the longer the half-life, the lower the radioactivity and thus the lesser probability of harm.
Along with a smattering of these long-lived isotopes, used fuel also has a collection of short-lived fission products (FPs), newly- minted atoms that are too small to fission. They form when big fuel atoms split in two, and some of the resulting FPs are harshly radioactive.
Since fission product atoms are formed from much larger atoms, and since these humongous atoms are held together with an overabundance of neutrons, an FP will usually start life with several more neutrons than its elemental peers—radioactive cesium-137, for example, versus plain old cesium-133. Fission products shake off (radiate) this extra baggage so they can settle into their new life as smaller, normal atoms.
When fuel atoms split, the fission products that result tend to occupy two distinct regions of the Periodic Table. (Sorry, alchemists, but gold isn’t on the list; that’s not how the nuclear cookie crumbles.)
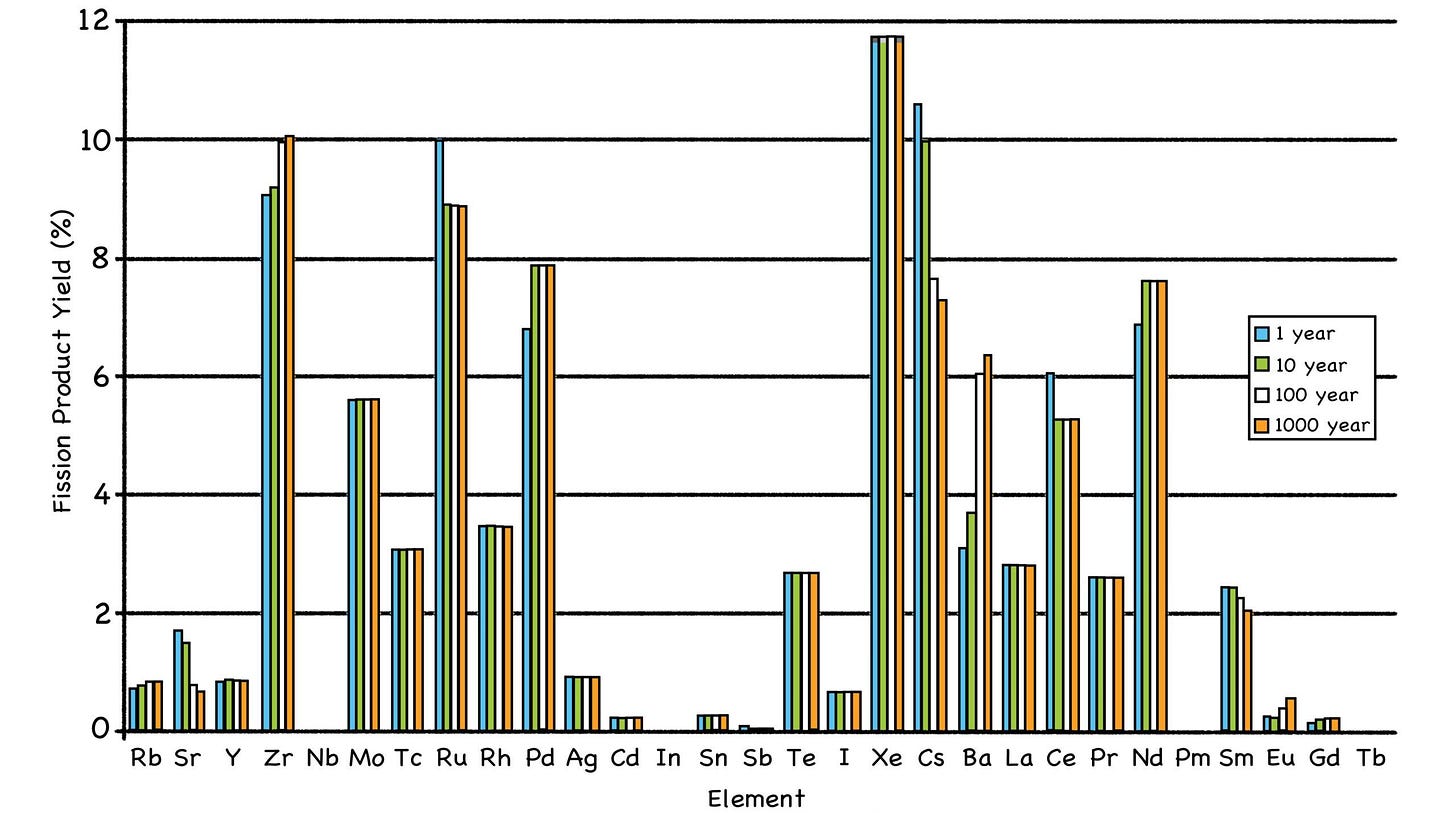
Fig. 33: Distribution of Fission Product Creation
Source: https://en.wikipedia.org/wiki/Fission_product_yield# /media/File:Fission_yield_volatile_2.png
The problem with FPs and TRUs forming in fuel pellets is that they’re pretty much evenly distributed throughout several tonnes of (very) mildly radioactive U-238 filler, which constitutes about 95% of the typical fuel pellet (Fig. 31). This is why all used fuel is treated as long-term radioactive waste. Although, considering the amount of energy produced per kilo of fissioned fuel, the volume of used fuel is downright minuscule, even from reactors that only burn 3% of their total fuel load (Figs. 31 and 34).
The good news is that both used fuel and depleted uranium can be recycled and thoroughly fissioned in fast-neutron reactors. This is different than reprocessing used fuel for another round in slow- spectrum LWRs, which for all their stellar performance are inefficient fuel burners. The unmoderated neutrons in a fast reactor can break down (think: “compost”) the 100,000-year transuranic waste that forms in used fuel into 300-year fission-product waste, producing gigawatts of power in the process. [4]
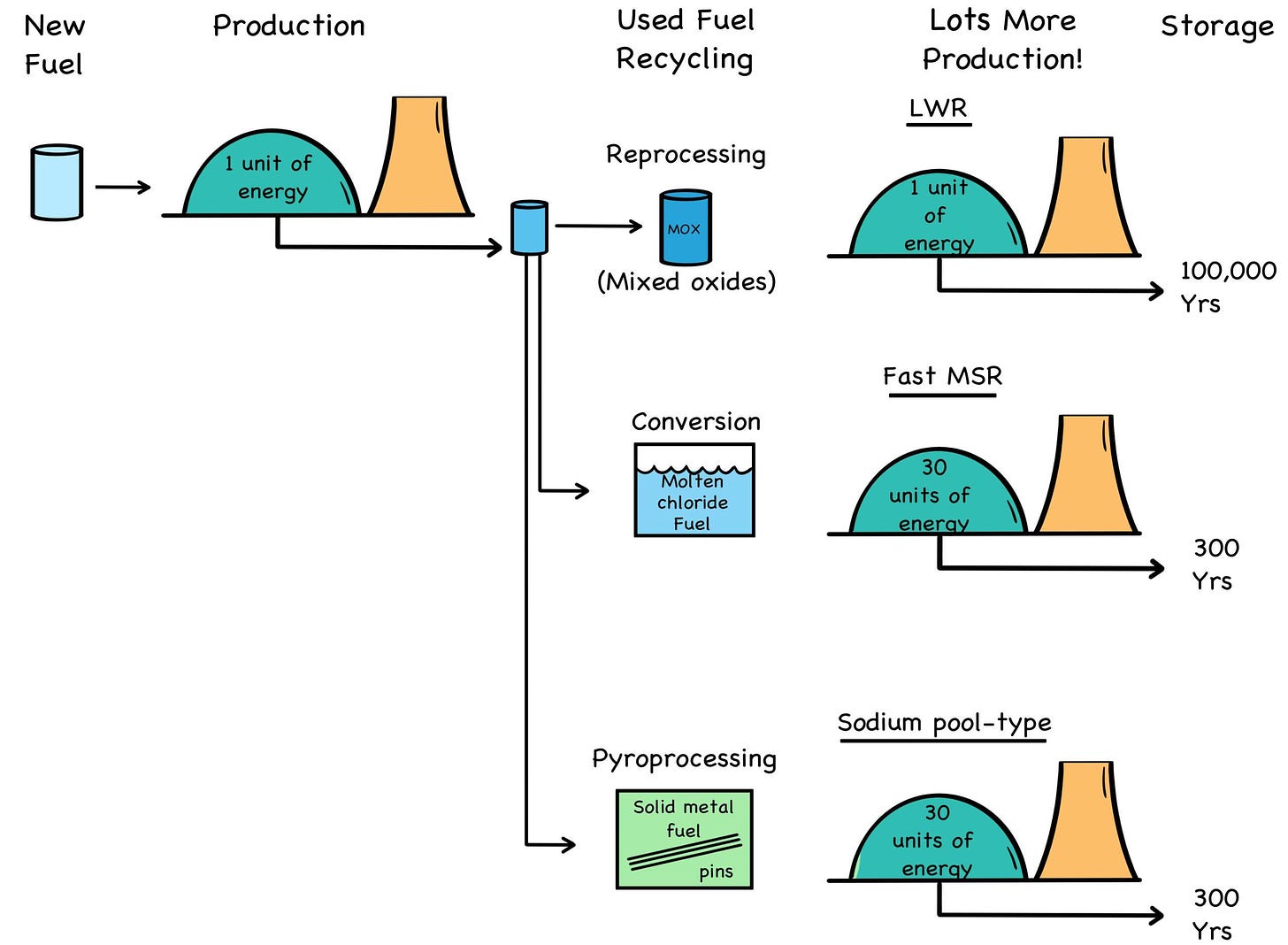
Fig. 34: Waste Reduction
Credit: By the authors
By using fast-neutron reactors, the US would have enough “spent” nuclear fuel for about 180 years of electricity at the nation’s current rate of consumption. [5] However, we should note that pro- cessing and recycling used fuel for complete burnup in fast reactors would be considerably more complicated, and expensive, than using depleted uranium.
5.3 DEPLETED, BUT FAR FROM SPENT
Depleted uranium (DU) is the leftover material that results from the solid-fuel enrichment process for light-water reactors. Several batches of natural uranium yellowcake must be depleted of their uranium-235 to enrich a single batch, which is then made into fuel pellets. The depleted uranium from which the U-235 has been separated is currently regardedas waste, but our nation’s stockpile of DU is actually a treasure trove of free fuel for fast reactors. However, there’s just one hitch:
You need eight times as much fissile material to kick-start a new fast-neutron reactor, than you need for a new slow-neutron reactor. This is because the faster a neutron flies, the smaller every fissionable target nucleus seems to be. This means a fast reactor needs a lot more neutrons to get the party started.
NERD NOTE: From the viewpoint of a zooming neutron, the perceived size or “cross-section” of a target nucleus is largely determined by the speed of said neutron, and is measured in “barns.” The term comes from the wry observation that if a neutron is going slow enough, every target nucleus will seem to be as big as a barn.
To a fast neutron, however, a target nucleus may seem to be only one barn wide, rather than its actual width of eight barns. This of course makes the target eight times smaller and thus eight times harder to hit.
The solution is to throw eight times more neutrons at the target. Thus, the initial fissile load for a fast reactor is about eight times the startup load of a slow-spectrum reactor like the LWR.
Another way of looking at it—you’ll need eight bags of briquettes to fire up the grill. But once a fast reactor is up and running, it can gradu- ally start breeding more and more of its own fuel from an increasing diet of DU or used LWR fuel, which is about 95% depleted uranium-238 (Fig. 31). With proper care and feeding, a fledgling fast reactor will mature into a self-sustaining compost bin for nuclear “waste.”
Having to build a bonfire in order to have a weenie roast is why fast reactors should always have a slightly positive breeding ratio. That is, instead of being an “iso-breeder” that makes just enough fuel for its own use, and not a smidgen more, a fast breeder reactor would breed a little more fuel than it actually needs, and set it aside. Depending on the breeding ratio, there will eventually be enough set-aside fissile to kick- start another fast reactor—without mining, refining, and processing a humongous new 8x startup load. And not to worry, the stockpiled fissile material from a breeder will be a mix of “reactor-grade” plutonium and other actinides: Great for nuclear power but useless for nuclear weapons.
With fast breeders making extra startup fuel to power their own buildout, our depleted uranium “waste” could serve as a long-term supply of free fuel for a national fleet of fast reactors. We have hundreds of thousands of tonnes of the stuff—about five or six times more than all the light-water reactor fuel we have enriched since the 1950s. [6]
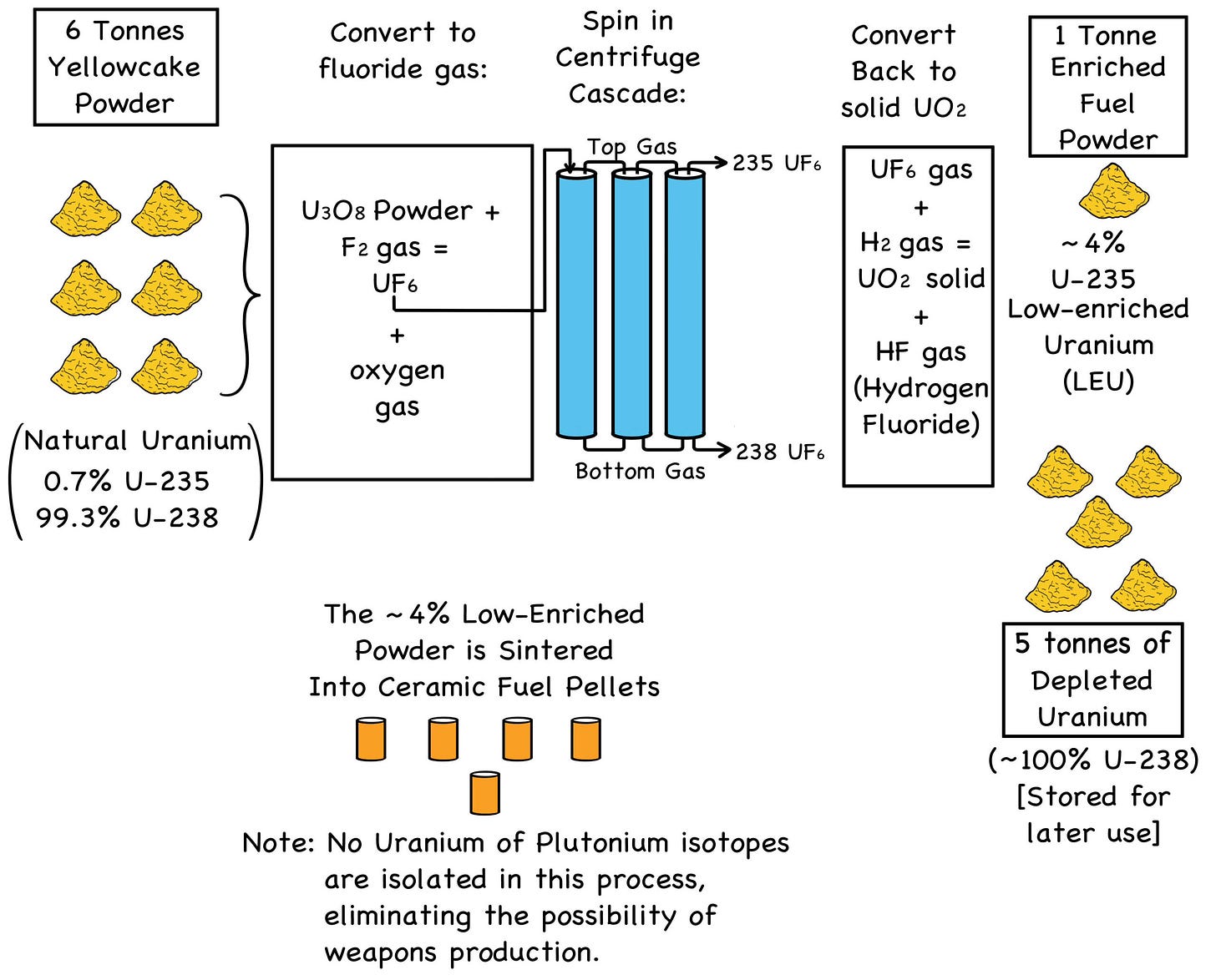
Fig. 34: Fuel Processing
Credit: By the authors
Unlike slow-neutron reactors, which tend to be picky eaters, fast reac- tors will eat everything they’re fed, and the mining has already been done.
NERD NOTE: Natural uranium contains only 0.7% of the scarce U-235 isotope fissioned in existing slow-neutron reactors. To make a batch of low-enriched uranium (LEU) to fuel a nuclear power plant, several batches of yellowcake powder are converted to uranium hexafluoride gas (UF6) and spun in a cascade (sequence) of high-speed centrifuges.
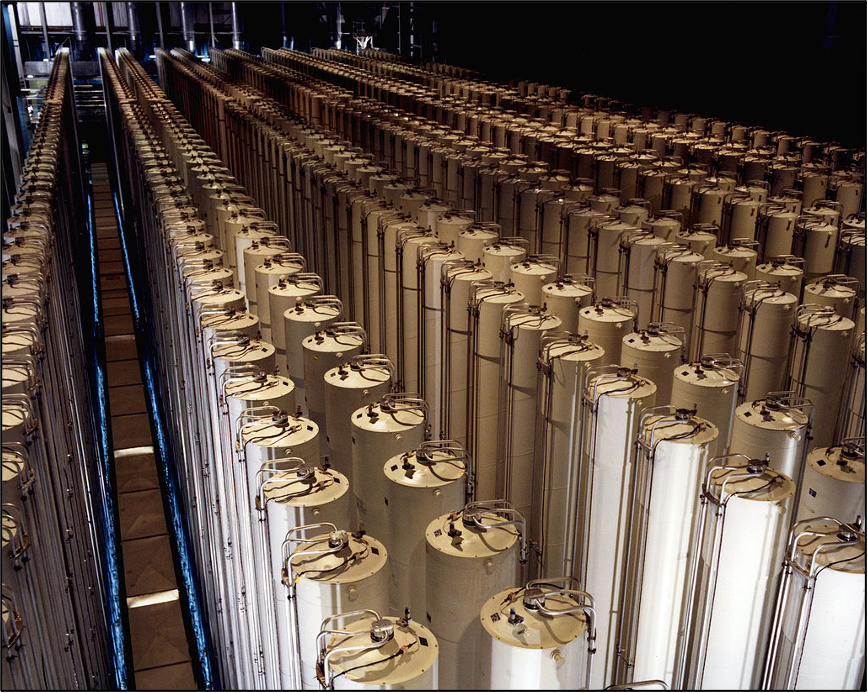
(Pg. 77) Centrifuge Cascade
Credit: US Nuclear Regulatory Commission (CC-BY-SA-2.0)
Spinning at 50,000 rpm, a centrifuge separates the uranium hexafluoride gas ever so slightly, with the heavier U-238 gas tending to drift toward the outside. The lighter U-235 gas tends to remain a bit closer to the center, and being a bit lighter, it will also tend to rise toward the top.
The “top gas” is drawn off and fed to the next centri- fuge, and so on down the cascade, thousands of times over, with a tiny bit of separation (and thus enrichment) in each centrifuge.
When the desired enrichment level is finally reached, the U-235 top gas and U-238 bottom gas are chemically converted back into separate batches of solid uranium oxide (a mix of UO2 and U3O8).
The enriched yellowcake powder made from the top gas is then “sintered” into fuel pellets—applying dry heat and pres- sure to form a dense, insoluble ceramic. The depleted uranium powder made from the bottom gas is set aside as waste.
Since DU is nearly pure uranium-238, it’s only slightly radioactive and can be easily transported on public highways. However, regulations have made it nearly impossible to transport more-radioactive used fuel to either a recycling facility or to a deep geologic repository (DGR). This overabundance of caution is in spite of the long-demonstrated safety of transport casks, which can withstand the direct impact of a rocket-assisted locomotive. (See the videos: [7])
It should be noted that when used fuel is transported to a repro- cessing facility, recycling this “spent” nuclear fuel into another round of reactor fuel requires sophisticated shielding and handling, both of which are expensive. So while either DU or SNF can be used to generate power in a fast reactor, depleted uranium is a lot less hassle all around. And like any country that enriches uranium, the US has a lot more DU than used fuel.
Indeed, after processing low-enriched reactor fuel for more than sixty years, the US has accumulated about 750,000 tonnes of depleted uranium. If this was fissioned in a fleet of fast reactors, it could power the nation for more than 1,500 years at our current rate of electricity consumption, or about 600 years when we transition to an all-electric society. (See our supplement on DU reserves: [8])
Don’t take our word for it. Here’s how climate scientist James Hansen puts it:
“With a fourth generation of nuclear power [Gen-IV reactors], you can have a technology that will burn more than 99 percent of the energy in the fuel. It would mean that you don’t need to mine uranium for the next thousand years.” [9]
In the long run, we think DU will prove to be more popular than SNF. As we see it, the widespread recycling of used LWR fuel will probably be done only if the public insists. And to be fair, the public just might insist, because thoroughly recycled used fuel would produce gigawatts of clean power, while also reducing its radioactive longevity from 100,000 years or more, to 300 years or less.
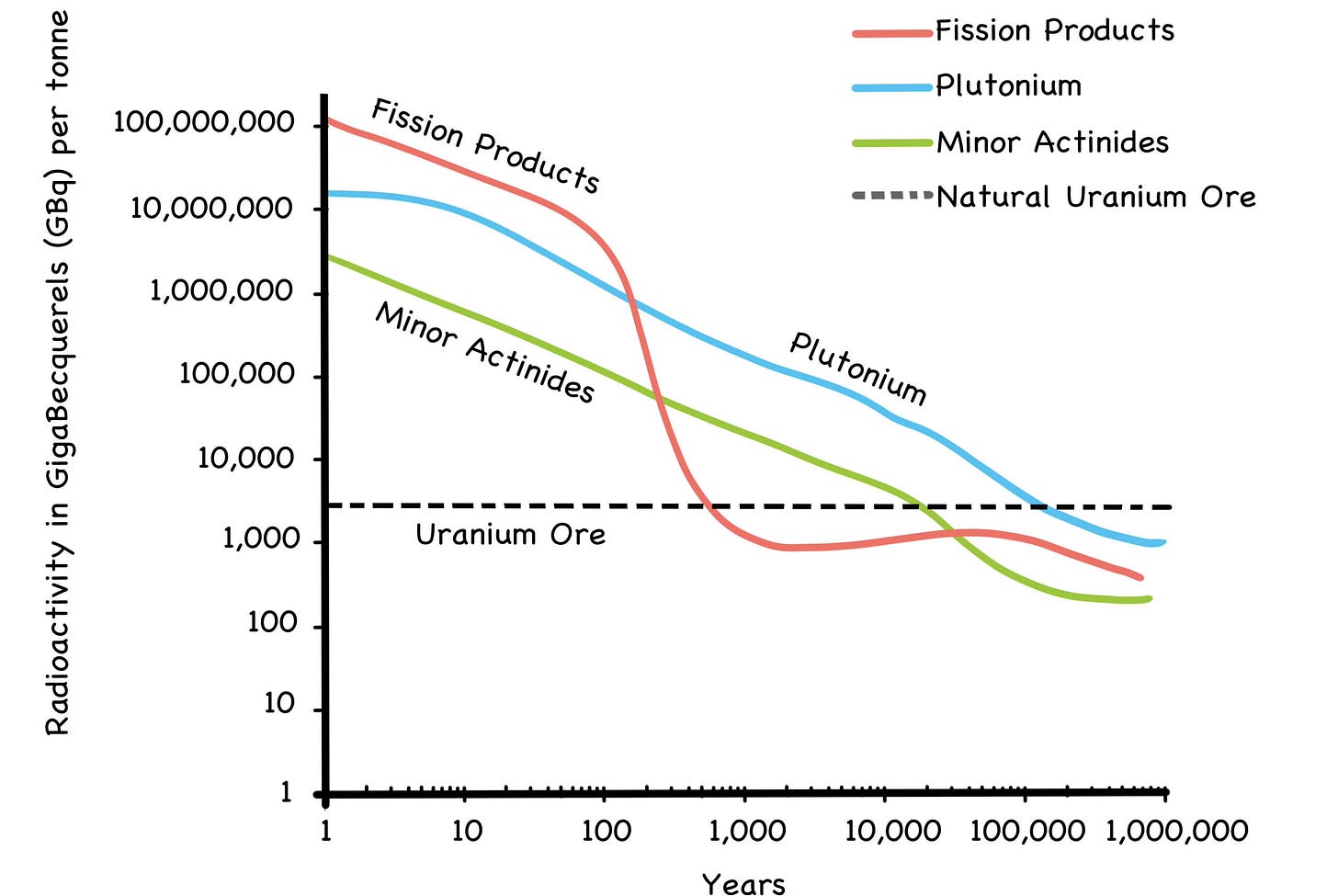
Fig. 36: Decay of Used Fuel to Natural Levels
Source: Thorium: Energy Cheaper Than Coal (2012) by Robert Hargraves, pg. 206
In any case, the radioactivity in used fuel is not the looming, rectify-or-die threat to humanity it’s often made out to be. It is true that the half-life of plutonium-239 is 24,000 years, and as we saw in Chapter 3 it takes ten half-lives to render a radioactive material harmless. This means the Pu-239 in used fuel is considered radioac- tive for 240,000 years.
At first glance, this might seem like a showstopper for nuclear power all by itself, but the hazard window for human health and safety is significantly shorter, despite any fearmongering to the contrary. In fact, after just 600 years, all penetrating gamma radiation is gone. From that point forward, you would have to literally grind the stuff up and inhale it or ingest it for the remaining alpha and beta radia- tion to do you any harm. [10] After 1,000 years, the radioactivity of used fuel will be no more intense than the mine from whence it came.
If scavengers in some far-flung future ever do go through the trouble of exhuming used fuel from an ancient repository, they would also need nuclear chemists and engineers to process and refine the stuff into any sort of significant threat. And if they had the technology to do that, they would also have the technology to make plutonium in a production reactor from a bit of yellowcake (see Chapter 19), and save themselves all the excavation and chemistry.
Like most scare stories about nuclear power, the “unsolvable problem of nuclear waste” isn’t a problem at all—unless it’s been made into one.
Learn More About Earth is a Nuclear Planet Here
Pre-Order the Book Today!